A dehydrated space-weathered skin cloaking the hydrated interior of Ryugu: research from the Hayabusa2 initial analysis team published in “Nature Astronomy”.
December 20, 2022 (JST)
Japan Aerospace Exploration Agency
Kyoto University
Kyushu University
Osaka Metropolitan University
Hiroshima University
Japan Agency for Marine-Earth Science and Technology
Tohoku University
Hokkaido University
The University of Tokyo
The Japan Aerospace Exploration Agency (JAXA) is conducting the first analysis of the sample returned from asteroid Ryugu by the Hayabusa2 mission with the Hayabusa2 Initial Analysis Team, comprising of six sub-teams and two Phase-2 curation institutes. Results from the Initial Analysis Team’s “Sandy Materials Analysis Team” have been published in the UK Journal “Nature Astronomy” on December 20, 2022 (JST).
Paper title:A dehydrated space-weathered skin cloaking the hydrated interior of Ryugu
Journal:Nature Astronomy
DOI:10.1038/s41550-022-01841-6
The initial analysis of the asteroid Ryugu samples
The sample from asteroid Ryugu was returned to Earth by the Hayabusa2 spacecraft on December 6, 2020. The initial description of the sample (Phase-1 curation) followed at the facility established at JAXA’s Institute of Space and Astronautical Science (ISAS). Parts of the sample were then distributed to the Hayabusa2 Initial Analysis Team, which consists of six sub-teams and two Phase-2 curation institutes. This division into specialized sub-teams is designed to achieve the scientific objectives of Hayabusa2 through high-precision analysis of different aspects of the sample. Data from the Phase-2 curation institutes’ comprehensive analysis of the grain characteristics will be included in the JAXA Hayabusa2 grain catalogue for future analysis proposals. Reports from the six initial analysis teams and Phase-2 curation institutes are being announced individually as the results are published in scientific journals. After all the results of the initial analysis have been announced, an overall summary is planned.
[Attachment]
A dehydrated space-weathered skin cloaking the hydrated interior of Ryugu
1. Key points
●
It has been already revealed by other analysis research groups [1-5] that asteroid Ryugu is composed of materials that have experienced severe aqueous alteration. However, the in-situ observations by the Hayabusa2 spacecraft were interpreted as the ancestor body of asteroid Ryugu having been dehydrated by thermal metamorphism (intense whole-asteroid-scale heating) after aqueous alteration [6], or that Ryugu was in an orbit that passed close to the Sun so that the asteroid’s surface was dehydrated to a depth of about 1 meter by solar irradiation [7]. This study resolves the apparent contradiction between the laboratory and in-situ results.
●
The surfaces of airless bodies are gradually altered due to several causes. The gradual alteration is called space weathering. This study showed that the surface of Ryugu has been altered by space weathering. However, asteroid Ryugu contains abundant hydrated phyllosilicates (a kind of clay), which are basically absent in the Moon and asteroid Itokawa. Due to these abundant phyllosilicates, space weathering found on the surface of Ryugu grains is unique, differing from the waterless Moon and Itokawa. So to speak, every airless body experiences different types of “sunburn” according to the character of each body.
●
The most characteristic feature of the space weathering of Ryugu is a higher abundance of grains covered by a few-µm thick silicate melt. Due to the melting by micrometeoroid impact, phyllosilicates on the surfaces of grains were dehydrated. The surface of Ryugu reflected the sunlight as if the entire body had experienced global thermal metamorphism due to a high abundance of grains covered by silicate melts on the surface of Ryugu.
●
There are many C-type asteroids in the main-belt of the asteroid belt. This study will play an important role in clarifying the actual state of space weathering and interpreting the reflectance spectra of water-bearing asteroids, including C-types.
2. Overview
As mentioned in the previous section, micrometeoroids (Note 1) impact on the surfaces of airless bodies with a speed of >10 km/s. The surfaces are also irradiated by the solar wind (a plasma flow from the Sun: Note 2) and solar and galactic cosmic rays (Note 3). These agents gradually alter surface chemical compositions, structures, and optical properties. This is called space weathering. The near-Earth asteroid (162173) Ryugu belongs to the class of C-type asteroids, which are the most abundant asteroid type. The samples returned from Ryugu allowed us to investigate the features of this C-type asteroid in laboratories. Space weathering has previously been investigated using the samples returned from the Moon and the S-type (rich in silicate minerals: Note 4) asteroid Itokawa [e.g. 8-14]. However, these samples are composed of anhydrous minerals. In contrast, the parent body of Ryugu (from which Ryugu is thought to be a fragment of reaccumulated material: Note 5) experienced severe aqueous alteration, the reaction between anhydrous minerals and water. Therefore, Ryugu contains abundant phyllosilicates (Note 6), a kind of clay. Ryugu grains on which space weathering was detected contain amorphized (where the orderly crystal arrangement of atoms is disturbed: Note 7) phyllosilicates and/or glassy surfaces with many burst vesicles. In both cases, Fe3+ ions in phyllosilicates were reduced to Fe2+ in the space-weathered surfaces and hydroxyl groups in phyllosilicates were lost, which means that water molecules were removed from the surface of Ryugu grains. In particular, the effects of dehydration were quite remarkable. These results indicate that dehydration of phyllosilicates on the surface of Ryugu plays an important role in the space weathering of C-type asteroids. A weak 2.7-µm absorption band obtained by the near-infrared spectrometer equipped on the Hayabusa2 spacecraft indicates the low abundance of hydroxyl groups on the surface. It is therefore possible that main-belt C-type asteroids that show a weak 2.7-µm band may also have experienced dehydration induced by space weathering rather than global thermal metamorphism.
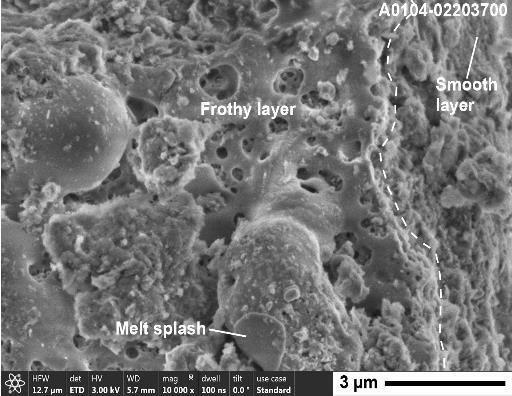
© Noguchi et al. (2022)
Fig. 1
Space weathering of a Ryugu grain. The smooth layer on the right side of the dotted line has been subjected to space weathering from solar wind irradiation. The frothy layer visible on the left side has experiences space weathering from micrometeoroid impacts, and contains deposits of thin layers of melted rock. This backscattered electron image allows us to read the complex history of Ryugu.
3. Summary of the main text
The major two factors of space weathering acting on airless bodies are solar wind irradiation and high-speed micrometeoroid impact. However, the degree to which these two factors affect and what effects they produce differ greatly depending on the materials of each airless body. Solar wind irradiation causes partial to perfect amorphization (Note 8) of the surface material, and the formation of nanometer-sized metallic iron [e.g. 8-15]. In addition, nanometer-sized metallic iron is formed in the glassy material condensed onto the surface of grains [8]. These facts were revealed by the studies of lunar and Itokawa samples.
However, it was not revealed whether nanometer-sized metallic iron is formed by space weathering in the case of dark asteroids such as C- and D-types or not. In addition, it is also unclear whether nanometer-sized metallic Fe affects the features of the reflectance spectra of C-type asteroids. Recently, there have been experimental studies of space weathering using CM chondrites, which are thought to originate from C-type asteroids. However, we have no certainty at all about the effects of space weathering of C-type asteroids [16-21]. Our team therefore had the first opportunity to provide factual evidence on the change in reflectance spectra (intensity of light reflected from the asteroid at different wavelengths that depends on material properties: Note 8) due to space weathering of C-type asteroids.
We initially began our study by seeking surface modifications to the grains from asteroid Ryugu that may be related to space weathering. As has been described in previous research [5], the Ryugu samples are very fragile. We faced difficulties in finding the space-exposed surfaces from the allocated samples because the grains had been already broken due to large differences between day- and night-time temperatures of more than 100 K [22], the shock when they were collected, and the shock during the entry processes to the Earth. Although the total amount of the allocated sample is only 0.7 mg, we had many tiny grains. By observing >500 grains collected at the touchdown #1 site with an average diameter of 70 µm, and >300 grains collected at touchdown site #2 with an average diameter of 50 µm, we found that about 6% of the observed grains show detectable surface modifications related to space weathering. The majority of the grains are fragments of much larger grains. To compare the space-weathering of the fine-grained samples with that of the large grains, we were able to observe the space-weathering textures of some large grains that were borrowed from the “Chemistry” and the “Stone” teams. We also investigated non-space-weathered grains. The result is consistent with the results of other teams: the samples recovered from Ryugu is very similar to CI chondrites. In other words, there are no differences in the mineralogy of petrology between small and large grains. As described in the next section, we found smooth and frothy layers.
(1) Space weathering layer found in the Ryugu particles (Part 1): Smooth layer
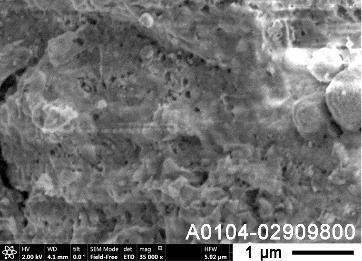
© Noguchi et al. (2022)
Fig. 2
Space weathering induced by solar wind irradiation (Smooth layer). The typical texture here shows holes that are considerably smaller than 1 µm (black appearance in the image), scattering on a smooth surface. Secondary electron image.
A smooth layer has a literally smooth surface. As shown in Fig. 2, the surface is rounded and smooth, with some open vesicles much less than 1 µm across. The smooth layers occupy ~5% of the grains collected at the touchdown #1 site and ~1% of those collected at the touchdown #2 site. To assess the formation mechanism of the smooth layers, we performed low-energy He+ ion irradiation experiment on the non-space-weathered Ryugu grains to simulate solar wind irradiation at JAXA. The textural modification before and after the irradiation experiment is shown in Fig. 3. After the experiment, a texture very similar to the smooth layer was formed.
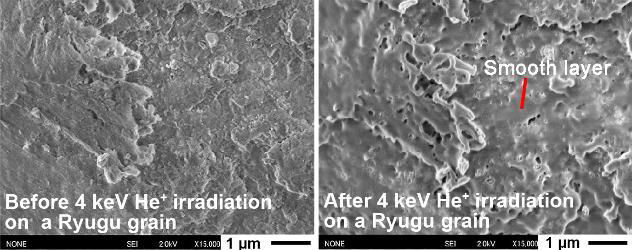
© Noguchi et al. (2022)
Fig. 3
Reproduction of smooth layer using a Ryugu grain by 4 keV He+ ion irradiation experiment. Secondary electron images.
The product of the irradiation experiment has internal textures similar to that of the smooth layers (Fig. 4). Electron diffraction patterns (Note 9) that are labeled as #1 in Fig. 4 show that the phyllosilicates in the continuous surface layer with a thickness of about 0.1 µm are amorphous. By contrast, crystal structures of the phyllosilicates below the continuous amorphous layers keep their crystal structures. Based on the textural and structural similarities, we interpreted that the smooth layers were space-weathering textures that were formed by the radiation damage of solar wind.
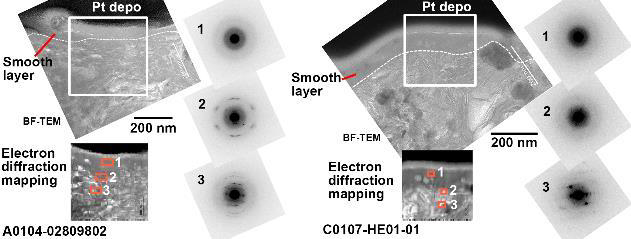
© Noguchi et al. (2022)
Fig. 4
Comparison of the cross-sectional textures of the natural smooth layer (left-hand images) and the product of the He ion irradiation experiment (right). In both cases, the layer above the white dotted line shows where the crystal structure has broken to form an amorphous smooth layer seen from the surface. On the right side of each image, three electron diffraction patterns are shown. In both cases, #1 indicates an amorphous structure, but #2 and #3 show dots indicating a crystal structure. HAADF-STEM images and electron diffraction patterns.
(2) Space weathering layer found in the Ryugu particles (Part 2): Frothy layer
Frothy layers were formed by the melting of the phyllosilicates and the release of volatiles during melting (Fig. 5 left). The frothy layers were found among ~1% of grains collected at the touchdown #1 site and ~2% of grains collected at the touchdown #2 site. Figure 5 right shows the surface of Murchison CM chondrite that was irradiated by pulse lasers. Although CM chondrites are different from CI chondrites, which are very similar to Ryugu samples, their surface is similarly melted and bubbling. Their internal textures are also very similar to each other (Fig. 6). Both Ryugu samples and Murchison CM chondrites are rich in phyllosilicates. When the phyllosilicates were heated instantaneously, water vapor was released during the melting of phyllosilicates (Note 10). The textural features indicate that the frothy layers were formed by pulse heating and melting of the surfaces of Ryugu grains.
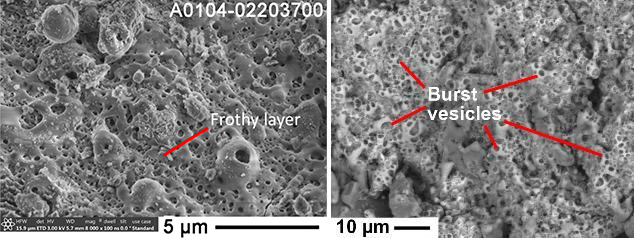
© Noguchi et al. (2022)
Fig. 5
Comparison of the surface textures of the natural frothy layer on a Ryugu grain (left) and that of the product of the laser irradiation experiment on Murchison CM chondrite (right). SE and BSE images.
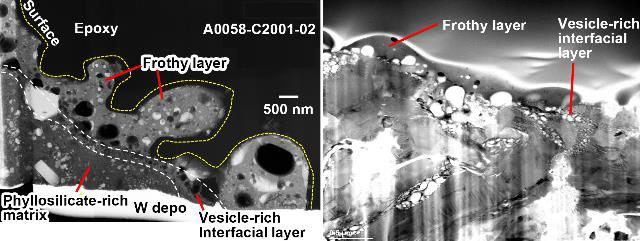
© Noguchi et al. (2022)
Fig. 6
Comparison of the cross-sectional textures of the natural frothy layer on a Ryugu grain (left) and the product of the laser irradiation experiment on Murchison CM chondrite (right). HAADF-STEM (left) and BF-STEM (right) images.
(3) Reduction of iron ions within the space weathered layer
Abundant phyllosilicates that compose Ryugu grains contain Fe ions, that can be either Fe3+ and Fe2+ cations (Note 11). Compared with the non-space-weathered phyllosilicates, most Fe ions in both smooth and frothy layers are Fe2+, which means that the reduction of Fe3+ to Fe2+ occurred by space weathering. However, there are few nanometer-sized metallic Fe in Ryugu grains although such metallic Fe is common as space-weathering products in lunar and Itokawa samples [8-15].
(4) Dehydration due to space weathering
Dehydration of hydrous phases by space weathering is a key to reconciling the discrepancy between the in-situ measurement of the reflectance spectra of Ryugu and the analytical results of the returned samples. As already described in section (2), the volatile component had been released from the frothy layer due to the micrometeoroid heating and the melting of the phyllosilicates. Frothy layers have chemical compositions of a mixture of anhydrous silicate glass and Fe-Ni sulfides, which are two major components that form the layers. Phyllosilicates below the frothy layers were also dehydrated at least to the depth of 1-2 µm from the top surfaces. In addition, space weathering induced by solar wind irradiation also dehydrated the smooth layer incompletely.
(5) Many Ryugu grains have more melted surfaces compared to those from Itokawa
1-2% of the Ryugu grains investigated have melted surfaces (frothy layers). By contrast, 0.3% of the Itokawa grains investigated have melted surfaces. It is known that the maximum temperatures during pulse heating experienced by the materials with ~30% microporosity tend to be higher than those with low microporosity like Itokawa grains. We think that the observed Ryugu grains were melted by frictional heating when micrometeoroid(s) hit loose aggregates of grains with high microporosity.
(6) Accumulative effect of space weathering on asteroid Ryugu
Space-weathering layers formed by solar wind irradiation (smooth layers) are formed continuously. By contrast, space-weathering layers formed by melting from micrometeoroid impact (frothy layers) are formed almost instantaneously. It is likely that micrometeoroid impact occurs occasionally and randomly. By considering these things, we drew a schematic diagram of the accumulation of the effects of the two types of space weathering on a Ryugu grain (Fig. 7). In Fig. 7, solar wind particles (plus ions and electrons in a solar wind plasma) accumulate from the top surface to ~0.1-µm deep. The accumulated solar wind particles decompose the crystal structures of the phyllosilicates and cause the partial dehydration of phyllosilicates. These effects are the space weathering induced by solar wind and they form smooth layers. Occasional micrometeoroid impact melts the surface of Ryugu grains and the melt covers the surfaces of Ryugu grains partially or completely. These effects are space weathering induced by micrometeoroid impact and create the frothy layers. The frothy layers are almost completely dehydrated and the phyllosilicates below the frothy layers are also dehydrated up to at least a few µm deep.
The major two factors to cause space weathering are solar wind irradiation and micrometeoroid impact. They are common to all airless bodies. However, space weathering products are quite variable among bodies according to their constituent materials, the distance from the Sun, and the size of the bodies. The effect on the reflectance spectra by the smooth layers may be negligible due to their very thin thickness, ~0.1 µm. However, the frothy layers are likely to affect the reflectance spectra because the total thickness of the frothy layers (2-3 µm thick) and the dehydrated phyllosilicates below the frothy layers (a few µm thick) are comparable with the wavelengths of near-infrared lights. If there are abundant grains covered by frothy layers exist on the surface of Ryugu, it is expected that the surface exhibits reflectance spectra as if they were composed of materials that experienced global thermal metamorphism.
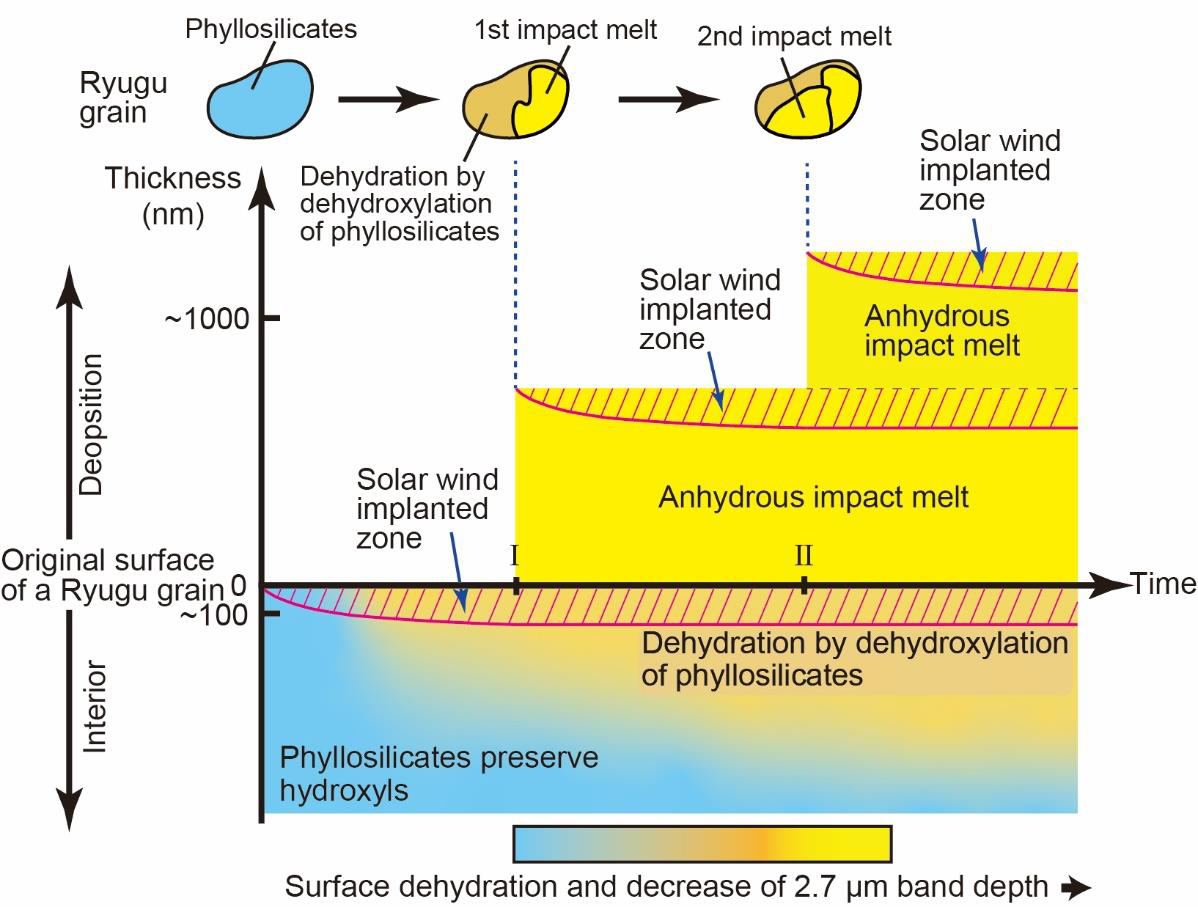
© Noguchi et al. (2022)
Fig. 7
Schematic diagram of the accumulation of the effects of space weathering on a Ryugu grain.
(7) Research significance for space weathering on C-type asteroids
C-type asteroids are most abundant among the main-belt asteroids that orbit the Sun between Mars and Jupiter. About 40% of these C-type asteroids do not have the absorption band that indicates the presence of hydroxyl base OH in their reflectance spectra. The absence has been interpreted as the result of dehydration by thermal metamorphism or as their constituent materials are similar to anhydrous interplanetary dust particles [24,25]. Based on our new results, we think that the dehydration by space weathering (especially that induced by heating from micrometeoroid impact) should be also taken into consideration.
4. Information of the article
Journal:
Nature Astronomy
Title:
A dehydrated space-weathered skin cloaking the hydrated interior of Ryugu
Authors:T. Noguchi1, T. Matsumoto2,1, A. Miyake1, Y. Igami1, M. Haruta3, H. Saito4,5, S. Hata6,7, Y. Seto8, M. Miyahara9, N. Tomioka10, H. A. Ishii11, J. P. Bradley11, K. K. Ohtaki11, E. Dobrică11, H. Leroux12, C. Le Guillou12, D. Jacob12, F. de la Peña12, S. Laforet12, M. Marinova13, F. Langenhorst14, D. Harries15, P. Beck16, T. H. V. Phan16, R. Rebois16, N. M. Abreu17, J. Gray18, T. Zega19, P-M. Zanetta19, M. S. Thompson20, R. Stroud21, K. Burgess22, B. A. Cymes23, J. C. Bridges24, L. Hicks24,25, M. R. Lee26, L. Daly26,27,28, P. A. Bland29, M. E. Zolensky30, D. R. Frank11, J. Martinez31, A. Tsuchiyama32,33,34, M. Yasutake35, J. Matsuno32, S. Okumura1, I. Mitsukawa1, K. Uesugi35, M. Uesugi35, A. Takeuchi35, M. Sun33,34,36, S. Enju37, A. Takigawa38, T. Michikami39, T. Nakamura40, M. Matsumoto40, Y. Nakauchi41, M. Abe41,42, M. Arakawa43, A. Fujii41, M. Hayakawa41, N. Hirata44, N. Hirata43, R. Honda45, C. Honda44, S. Hosoda40, Y. Iijima41*, H. Ikeda41, M. Ishiguro46, Y. Ishihara41, T. Iwata41,42, K. Kawahara41, S. Kikuchi47, K. Kitazato44, K. Matsumoto48,42, M. Matsuoka41, Y. Mimasu41, A. Miura41, T. Morota38, S. Nakazawa41, N. Namiki48,42, H. Noda48,42, R. Noguchi49, N. Ogawa41, K. Ogawa41, T. Okada41,42, C. Okamoto43*, G. Ono41, M. Ozaki41,42, T. Saiki41, N. Sakatani50, H. Sawada41, H. Senshu47, Y. Shimaki41, K. Shirai43, S. Sugita38,47, Y. Takei41, H. Takeuchi41,42, S. Tanaka41,42, E. Tatsumi51,38, F. Terui52, R. Tsukizaki41, K. Wada47, M. Yamada47, T. Yamada41, Y. Yamamoto41, H. Yano41,42, Y. Yokota41, K. Yoshihara41, M. Yoshikawa41,42, K. Yoshikawa41, R. Fukai41, S. Furuya41, K. Hatakeda53, T. Hayashi41, Y. Hitomi53, K. Kumagai53, A. Miyazaki41, A. Nakato41, M. Nishimura41, H. Soejima53, A. I. Suzuki53, T. Usui41, T. Yada41, D. Yamamoto41, K. Yogata41, M. Yoshitake41, H. C. Connolly, Jr.19,54, D. S. Lauretta19, H. Yurimoto55, K. Nagashima11, N. Kawasaki55, N. Sakamoto56, R. Okazaki57, H. Yabuta9, H. Naraoka57, K. Sakamoto41, S. Tachibana58, S. Watanabe59, Y. Tsuda41
1Division of Earth and Planetary Sciences, Kyoto University, Kitashirakawaoiwake-cho, Sakyo-ku, Kyoto 606-8502, Japan.
2The Hakubi Center for Advanced Research, Kyoto University, Kitashirakawaoiwake-cho, Sakyo-ku, Kyoto 606-8502, Japan.
3Institute for Chemical Research, Kyoto University, Gokasho, Uji, Kyoto 611-0011, Japan.
4Institute for Materials Chemistry and Engineering, Kyushu University, Fukuoka 816-8580, Japan.
5Pan-Omics Data-Driven Research Innovation Center, Kyushu University, Fukuoka 816-8580, Japan.
6Interdisciplinary Graduate School of Engineering Sciences, Kyushu University, Fukuoka 816-8580, Japan.
7The Ultramicroscopy Research Center, Kyushu University, Fukuoka 819-0395, Japan.
8Department of Geosciences, Osaka Metropolitan University, Sugimoto 3-3-138, Sumiyoshi-ku, Osaka 558-8585, Japan.
9Department of Earth and Planetary Systems Science, Hiroshima University, 1-3-1 Kagamiyama, Higashi-Hiroshima City, Hiroshima, 739-8526, Japan.
10Kochi Institute for Core Sample Research, X-Star, JAMSTEC, 200 Monobe Otsu, Nankoku, Kochi 783-8502, Japan.
11Hawai‘i Institute of Geophysics and Planetology, The University of Hawai‘i at Mānoa, 1680 East-West Road, POST Building, Room 602, Honolulu, HI 96822, USA.
12Université de Lille, CNRS, INRAE, Centrale Lille, UMR 8207-UMET-Unité Matériaux et Transformations, F-59000 Lille, France.
13Université de Lille, CNRS, INRAE, Centrale Lille, Université Artois, FR 2638-IMEC-Institut Michel-Eugène Chevreul, F-59000 Lille, France.
14Institut für Geowissenschaften, Friedrich-Schiller-Universität Jena, Carl-Zeiss-Promenade 10, 07745 Jena, Germany.
15European Space Resources Innovation Centre, Luxembourg Institute of Science and Technology, 41 rue du Brill, 4422 Belvaux, Luxembourg.
16Institut de Planétologie et d'Astrophysique de Grenoble (IPAG), CNRS, Université Grenoble Alpes, 38000 Grenoble, France.
17NASA Langley Research Center, Hampton, VA 23681-2199, USA.
18Materials Characterization Lab, The Pennsylvania State University Materials Research Institute, Millennium Science Complex, Pollock Road, University Park, PA16802, USA.
19Lunar and Planetary Laboratory, Department of Planetary Sciences, The University of Arizona, 1629 E. University Blvd., Tucson AZ 85721-0092, USA.
20Department of Earth, Atmospheric and Planetary Sciences, Purdue University, 550 Stadium Mall Drive, West Lafayette, IN 47907-2051, USA.
21Buseck Center for Meteorite Studies, Arizona State University, 781 E Terrace Road, Tempe, AZ 85281, USA.
22Materials Science and Technology Division, U.S. Naval Research Laboratory, Washington, DC 20375, USA.
23NRC Postdoctoral Research Associate, U.S. Naval Research Laboratory, Washington, DC 20375, USA.
24Space Park Leichester, The University of Leicester, 92 Corporation Road, Leicester, LE4 5SP, UK.
25School of Geology, Geography and the Environment, The University of Leicester, University Road, Leicester, LE1 7RH, UK.
26School of Geographical and Earth Sciences, The University of Glasgow, Molema Building, Lilybank Gardens, Glasgow G12 8QQ, UK.
27Australian Centre for Microscopy and Microanalysis, The University of Sydney, Sydney, New South Wales, Australia.
28Department of Materials, The University of Oxford, Parks Road, Oxford, OX1 3PH, UK.
29School of Earth and Planetary Sciences, Curtin University, GPO Box U1987, Perth, Western Australia 6845, Australia.
30ARES, NASA Johnson Space Center, 2101 NASA Parkway, Houston, Texas 77058, USA.
31Jacobs Engineering, 1999 Bryan Street, Suite 1200, Dallas, Texas 75201, USA.
32Research Organization of Science and Technology, Ritsumeikan University, 1-1-1 Nojihigashi, Kusatsu, Shiga 525-8577, Japan.
33CAS Key Laboratory of Mineralogy and Metallogeny, Guangdong Provincial Key Laboratory of Mineral Physics and Materials, Guangzhou Institute of Geochemistry, Chinese Academy of Sciences (CAS), Guangzhou 510640, China.
34CAS Center for Excellence in Deep Earth Science, Guangzhou 510640, China.
35Japan Synchrotron Radiation Research Institute, 1-1-1 Kouto, Sayo-cho, Sayo-gun, Hyogo 679-5198, Japan.
36University of Chinese Academy of Sciences, Beijing 100049, China.
37Department of Mathematics, Physics, and Earth Science, Ehime University, 2-5 Bunkyo-cho, Matsuyama, Ehime 790-8577, Japan.
38Department of Earth and Planetary Science, The University of Tokyo, 7-3-1 Hongo, Bunkyo-ku, Tokyo 113-0033, Japan.
39Faculty of Engineering, Kindai University, Hiroshima Campus, 1 Takaya Umenobe, Higashi-Hiroshima, Hiroshima 739-2116, Japan.
40Department of Earth Science, Tohoku University, 6-3 Aoba, Aramaki, Aoba-ku, Sendai 980-8578, Japan.
41Institute of Space and Astronautical Science, Japan Aerospace Exploration Agency, 3-1-1 Yoshinodai, Chuo-ku, Sagamihara, Kanagawa 252-5210, Japan.
42The Graduate University for Advanced Studies, SOKENDAI, Hayama 240-0193, Japan.
43Department of Planetology, Kobe University, 1-1 Rokkodai-cho, Nada-ku, Kobe, Hyogo 657-8501, Japan.
44Aizu Research Center for Space Informatics, The University of Aizu, Ikki-machi, Aizu-Wakamatsu, Fukushima 965-8580, Japan.
45Department of Information Science, Kochi University, 2-5-1 Akebono-cho, Kochi 780-8520, Japan.
46Department of Physics and Astronomy, Seoul National University, Seoul 08826, Korea.
47Planetary Exploration Research Center, Chiba Institute of Technology, 2-17-1Tsudanuma, Narashino, Chiba 275-0016, Japan.
48National Astronomical Observatory of Japan, 2-21-1 Osawa, Mitaka, Tokyo 181-8588, Japan.
49Faculty of Science, Niigata University, 2-8050 Ikarashi, Nishi-ku, Niigata 950-2181, Japan.
50Department of Physics, Rikkyo University, 3-34-1 Nishiikebukuro, Toshima-ku 171-8501, Japan.
51Instituto de Astrofísica de Canarias, University of La Laguna, Tenerife, Spain.
52Department of Mechanical Engineering, Kanagawa Institute of Technology, Atsugi 243-0292, Japan.
53Marine Works Japan Ltd., 3-54-1, Oppamahigashi-cho, Yokosuka 237-0063, Japan.
54Department of Geology, School of Earth and Environment, Rowan University, Glassboro, NJ 08028, USA.
55Department of Earth and Planetary Sciences, Hokkaido University, Kita-10 Nishi-8, Kita-ku, Sapporo 060-0810, Japan.
56Creative Research Institution Sousei, Hokkaido University, Kita-21, Nishi-10, Kita-ku, Sapporo 001-0021, Japan.
57Department of Earth and Planetary Sciences, Kyushu University, 744 Motooka, Nishi-ku, Fukuoka 819-0395, Japan.
58UTokyo Organization for Planetary and Space Science, The University of Tokyo, 7-3-1 Hongo, Bunkyo-ku, Tokyo 113-0033, Japan.
59Department of Earth and Environmental Sciences, Nagoya University, Furo-cho, Chikusa-ku, Nagoya 464−8601, Japan.
*Deceased.
DOI No.:10.1038/s41550-022-01841-6
5. Notes
(Note 1) Meteoroids are natural solid materials that move at high speed in interplanetary space. Meteoroids larger than 2mm that pass through the Earth’s atmosphere and fall to the Earth’s surface are called meteorites. Smaller meteoroids are called micrometeoroids. Although we do not know the sizes of meteoroids, in this case, we have referred to them as micrometeoroids.
(Note 2) A gas decomposed into positive ions and electrons due to high temperatures.
(Note 3) Solar cosmic rays are high-energy charged particles from the Sun, which are emitted during solar flares and coronal mass ejection. The typical kinetic energies of solar wind particles are a few keV. By contrast, solar cosmic rays' energy is from MeV to GeV. Galactic cosmic rays are high-energy particles formed outside the Solar System. Typical kinetic energies range from 100 MeV to 1 GeV.
(Note 4) S-type asteroids are rocky asteroids containing abundant silicate minerals.
(Note 5) Ryugu is a rubble-pile asteroid, which is a loose aggregate composed of fragments formed by the destruction of a larger body.
(Note 6) Ryugu contains two types of phyllosilicate minerals: saponite and serpentine. The former contains inter-layer water molecules in the crystal. The water molecules can come in and out easily from the crystal. However, saponite in the returned samples from Ryugu has already lost the inter-layer water molecules while on the surface of Ryugu. Please read reference [2] for details.
(Note 7) A reflectance spectrum displays the intensities of reflected lights as the function of wavelength (or wavenumber).
(Note 8) Due to the amorphization of a crystal, the ordered three-dimensional arrays of atoms or ions in a crystal are disturbed and their regularity is lost.
(Note 9) Because electrons have also the character of waves, electron beams interfere with the ordered arrays of atoms or ions in crystals. Characteristic interference patterns formed are called electron diffraction patterns.
(Note 10) For example, due to heating, serpentine Mg6Si4O10(OH)8 is decomposed into 3Mg2SiO4+SiO2+4H2O↑ . In this dehydration decomposition, water molecules (water vapor) are emitted.
(Note 11) This result is obtained from the analyses of the iron X-ray absorption near-edge fine structures using an electron energy loss spectrometer equipped with a scanning transmission electron microscope and synchrotron radiation facilities.
6.References
[1] Yada, T. et al. Nature Astron. 6, 214–220 (2021).
[2] Yokoyama, T. et al. Science 10.1126/science.abn7850 (2022).
[3] Nakamura, E. et al. Proc. Jpn. Acad. Ser. B 98, 227–282.
[4] Ito, M. et al. Nature Astron. https://doi.org/10.1038/s41550-022-01745-5 (2022).
[5] Nakamura, T. et al. Science 10.1126/science.abn8671 (2022).
[6] Kitazato, K. et al. Nature Astron. 5, 246–250 (2021).
[7] Morota, T. et al. Science 368, 654–659 (2020).
[8] Keller, L. P. & McKay, D. S. Geochim. Cosmochim. Acta 61, 2331–2341 (1997).
[9] Noble, S., Pieters, C. M. & Keller, L. P. Icarus 192, 629–642 (2007).
[10] Noguchi, T. et al. Science 333, 1121–1125 (2011).
[11] Noguchi, T. et al. Meteorit. Planet. Sci. 49, 188–214 (2014).
[12] Matsumoto, T. et al. Icarus 257, 230–238 (2015).
[13] Thompson, M. S. et al. Earth Planet. Space 66, 89 (2014).
[14] Burgess, K. D. & Stroud, R. J. Geophys. Res. Planets 123, 2022–2037 (2018).
[15] Hicks, L. et al. Meteorit. Planet. Sci. 55, 2599–2618 (2020).
[16] Thompson, M. S. et al. Icarus 319, 499–511 (2019).
[17] Thompson, M. S. et al. Icarus 346, 113775 (2020).
[18] Matsuoka, M. et al. Astrophys. J. 890, L23 (12pp) (2020).
[19] Lantz, C. et al. Icarus 285, 43–57 (2017).
[20] Laczniak, D. L. et al. Icarus 364, 114479 (2021).
[21] Trang, D. et al. Planet. Sci. J. 3:68 (12pp) (2021).
[22] Okada, T. et al. (2020) Nature 579, 518–522 (2020).
[23] Hiroi, T. et al. Science 261, 1016–1018. (1993).
[24] Vernazza, P. et al. Astrophys. J. 806:204 (10pp) (2015).
[25] Watanabe, S. et al. Science 364, 268-272 (2019).